You are here
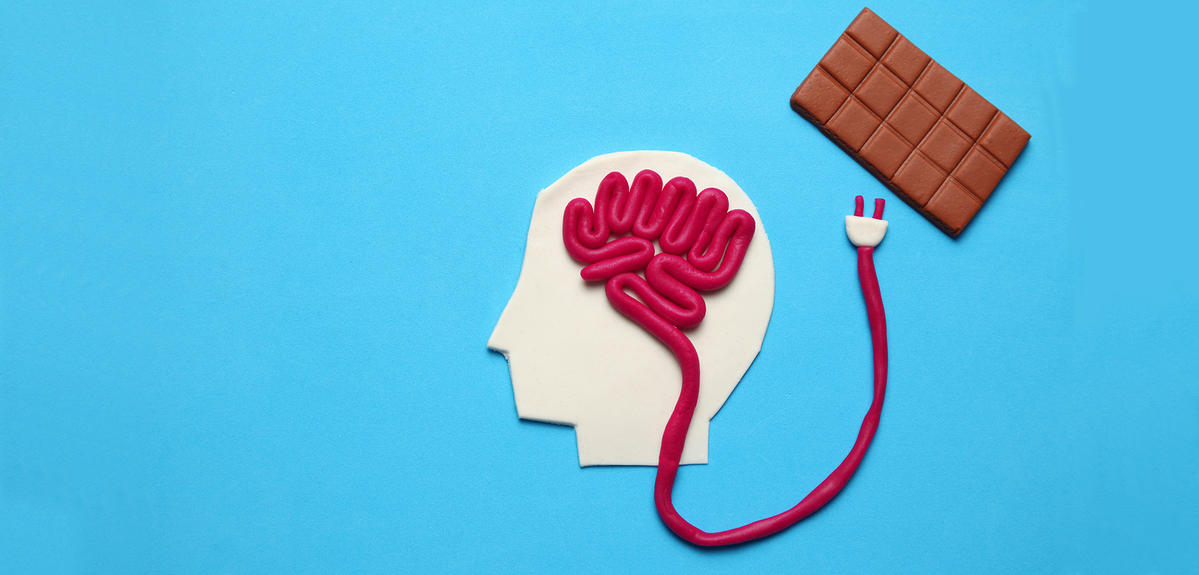
Eating is not just about digestion, the gut or the stomach; it is also, and most often, about hunger, disgust or greed. Unlike other essential functions such as breathing – which is continuous and mainly independent of any control – eating is a regular but occasional activity that we have the impression of consciously controlling and that is likely to give us pleasure. In short, deciding whether it is time to eat, or leave the table, to fancy one dish or another, is down to the brain. This organ therefore plays a key role in governing appetite by assembling the information that comes from our senses, memory, digestive system and entire body regarding what we are missing, what we would like and the nutrient content of what we have eaten.
Our dietary behaviour is indeed based on the expression of several independent brain circuits, some of which tend to act on desire and others on the need to eat. Understanding how they function or malfunction is therefore essential for clarifying and treating certain disorders that affect dietary behaviour and thus achieve more efficient control of the current obesity epidemic.
A wave of information from the gut to the brain
Under normal circumstances, we are not hungry all the time, a state that is referred to as satiety. It can last for hours and results from a flow of information from the digestive tract to the brain. “Satiety should not be confused with being satisfied,” explains Gilles Mithieux, research professor at the INSERM and head of the nutrition, diabetes and brain laboratory at the Université Claude Bernard in Lyon (southeastern France). “Being satisfied means no longer being hungry, a feeling triggered by signals from the digestive tract to the brain, which indicate that the stomach is full. Satiety, on the other hand, is the absence of hunger that follows a meal and will last until the next one. Its duration can vary, based on the content of the previous meal.”
But what are these indicators? Depending on the presence or not of food in the stomach, the brain will release signals that are orexigenic if they stimulate appetite or anorexigenic if they suppress hunger. Thus the central nervous system and brain conduct a dialogue via numerous chemical mediators such as cholecystokinine. “This peptide, which notably serves to release bile, also has the effect of reducing hunger by communicating with the brain,” explains Gilles Mithieux. However, the appetite suppressant effects of cholecystokinine vary according to individuals and their age. For example, while this mediator induces a drastic reduction in hunger in young male rats, it will have a much weaker anorectic effect in elderly or obese animals.
Following a meal, the stomach sends a flow of information to the brain via the vagus nerve. The intensity and frequency of this vagus nerve stimulation will depend on the type of foods ingested and play an important role in satiation. “Fats or sugars are very rapid appetite suppressants, but they do not reduce hunger in the longer term,” says Gilles Mithieux. “On the contrary, proteins or dietary fibres, which contain less energy, will delay it.” In fact, it is the ingestion of protein or fibre, and not sugar, that stimulates the vagus nerve! In this way, and within a few minutes, the brain can identify the quality of the meal ingested and modulate appetite.
From the mouth to the brain….
“Perceptions of the food on our plate start with the senses and the first chemical signals are released during mastication. For example, fatty acids are detected in the mouth and a message is sent to the brain,” explains Christophe Magnan, a university professor and head of the research team focusing on blood sugar regulation by the central nervous system at the Functional and Adaptive Biology Unit (BFA).1 Taste buds on the tongue thus recognise the presence of fatty acids via the CD36 receptor. Within seconds, the central nervous system is alerted to the ingestion of fat. “The rapid detection of fat in mammals could be explained from an evolutionary point of view, because weight-for-weight, lipids supply twice as much energy as carbohydrates. A craving for fat is therefore vital.”
As soon as digestion starts, changes to the blood levels of fatty acids are detected by specialised neurons in the hypothalamus. In rodents, it has been shown in the laboratory that an increase in fatty acids such as oleic acid is identified by hypothalamic neurons which, in return, alter dietary consumption. “Experiments have demonstrated that increasing the oleic acid content in the diet of rats reduced their food intake,” says Christophe Magnan. Inversely, by suppressing the action of CD36, the appetite suppressant effect of fatty acids is lost and “the rats rapidly become obese.”
The paradox of fatty acids
“This satietogenic potential will depend on the type of fatty acid released,” notes Christophe Magnan, who has studied the action of these molecules on the brain. During a meal, any fat ingested is burned or stored in the form of triglycerides in the adipose tissue. During the night or fasting, this stored fat is released into the bloodstream in the form of fatty acids. But if the latter have a considerable satietogenic potential, why are we hungry when we wake up in the morning? Specialists have been addressing this apparent nutritional paradox for many years. “The high levels of fatty acids measured when waking up in the morning should indeed suppress the desire to eat!” the researcher insists.
In fact, it has been shown that fatty acids arising from the “melting” of adipose tissue and those produced from triglycerides are not processed in the same way by the brain. “The hypothesis – which has now been confirmed – is that after a meal, circulating triglycerides are hydrolysed directly at the level of the brain.”


This cerebral metabolism is controlled by an enzyme in the hypothalamus: lipoprotein lipase. After a meal, this enzyme transforms recently ingested triglycerides into fatty acids. “Only these acids are involved in satiety, not those released during fasting,” the scientist points out. Experiments thus showed that when the production of lipoprotein lipase was blocked in mice, they were deprived of the appetite suppressant effect of the fat they had ingested and they tended to become obese.
It has since been found that this suppressant effect is not based on fat alone but could also be initiated by the ingestion of fibre in fruits and vegetables! Because this fibre cannot be digested by human enzymes, the micro-organisms in our gut microbiota take over. Indeed, these hosts transform dietary fibre into short-chain fatty acids that can induce a sensation of satiety. “Fibre is extremely important because it does not supply any energy directly, while at the same time producing short-chain fatty acids that exert an appetite suppressant effect,” Gilles Mithieux stresses.
Can intestinal glucose prevent obesity?
Around ten years ago, Gilles Mithieux and Christophe Magnan discovered another important mechanism regulating dietary behaviour: intestinal neoglucogenesis. “The intestine does not just absorb glucose, it can also produce it,” explains Gilles Mithieux. It was observed that two types of nutrients, proteins and dietary fibre, can trigger this synthesis of intestinal glucose. “The intestinal glucose from these foods acts as a messenger to inform the brain of the presence of fibre or protein in the gut, which in turn leads to a reduction in hunger and an improved regulation of blood sugar levels,” summarises the scientist.
This glucose protects against diabetes and obesity, as shown in mice that have been mutated to overexpress the enzyme that enables the production of intestinal glucose. “The result is that they put on half as much weight, even with a very fatty diet,” points out Gilles Mithieux. Until a molecule is found that can stimulate this glucose production, eating fibre and proteins appears to be a good way to prevent obesity and diabetes.

When the brain changes shape after a meal
Some groups of cerebral neurons are specifically responsible for inhibiting food intake; this is notably the case for POMC neurons. “Since 2004, we have known that this group is able to change the configuration of its connections as a function of nutritional status. This has been demonstrated in the brains of mice,” explains Alexandre Benani, a scientist at the Centre for Taste and Feeding Behaviour (CSGA)2 in Dijon (eastern France) and head of the Plasticity of Feeding Circuits team at the Dijon-based Université de Bourgogne Franche-Comté. Intense fasting or prolonged overeating will thus lead to a reconfiguration of the brain at the level of POMC neurons. This neuronal plasticity will modulate dietary behaviour and allow the body to best adapt to changing metabolic and nutritional conditions, while maintaining an energy balance as much as possible.
“We have seen that during a standard meal, the electrical activity of POMC neurons is tripled in the mouse,” says Alexandre Benani. Because the effect of POMC neurons is anorectic, their stimulation following a meal reduces food intake. The second observation is that a meal will modify the interactions between POMC neurons and neighbouring cells. “After fasting, these neurons are covered with astrocytes that supply them with nutrients, but after a meal these astrocytes will retract.” It is this change in the shape of astrocytes that will restart neuronal activity and stop hunger.
This retraction is induced by the blood sugar peak that follows a meal. “To demonstrate this, we used an antidiabetic drug that blocks the postprandial glycaemic peak. The result was that without any rise in blood sugar levels, the astrocytes did not retract and there was no increase in the activity of POMC neurons. By modifying mice to artificially trigger the retraction of their astrocytes, we managed to modify the number and size of their meals,” the researcher adds.
On the other hand, following a fast-food type (i.e. a lipid-rich) meal, neither POMC activation nor astrocyte retraction is observed. The fat therefore does not have the same type of appetite suppressant effect as glucose; in other words, the latter is indeed satietogenic but this depends on other neuronal circuits. “We know that satiety is a function of the type of nutrients ingested. Carbohydrates have a clearly better satietogenic effect than lipids. This study has thus provided a further neurophysiological explanation for the observations made by nutritionists,” Alexandre Benani sums up.
Are your eyes bigger than your stomach?
It remains that in human beings, starting or stopping to eat is not just a question of satiety and metabolic regulation. The human diet is based also, and above all, on pleasure. This is a so-called “hedonic” control that will condition the choice and frequency of our meals, as well as our food preferences.
“Depending on our senses – sight, taste and smell – we will stop eating or not. Although we may start a meal with pleasure, this epicurean sensation will not last. We then lay down our cutlery, or we move from the starter to the main dish,” explains Moustafa Bensafi, research professor at the Lyon Neuroscience Recherche Centre (CRNL).3 This balance between pleasure and lack of pleasure will govern how we eat.
This change to the hedonic value of a food is reflected in the brain. “If what you are eating is pleasant, it is the median orbitofrontal cortex that is stimulated. Its activity then declines as does our pleasure,” Moustafa Bensafi adds. “The lateral orbitofrontal cortex then takes over and induces restrictive behaviours”. In other words, after a few pieces of chocolate, the sensory pleasure is no longer the same, and this generally drives us to put the rest of the bar back in the cupboard. This is an essential function “because it allows us to have the most varied and balanced diet possible; without it, we would be eating all the time and always the same thing!”
A desire that we need
“There is a tendency to oppose eating as a vital need and eating for pleasure,” according to Serge Luquet, research professor and leader of the Central Control of Feeding Behaviour and Energy Expenditure (C2OFFEE) team at the BFA. The former brings into play our reptilian brain, in an active dialogue between the brain stem and the hypothalamus. “Pleasure”, on the other hand, is localised in the dopaminergic, or mesocorticolimbic network, “which projects from the centre of the brain towards the prefrontal cortex, the amygdala and the striatum, in particular”, explains Serge Luquet. This “reward” system is governed by the action of dopamine.
It involves three elements, starting with learning: “this is the classic Pavlov dog experiment. You see chocolate and before you have even tasted it, your brain releases dopamine because it has learned that it likes it,” the scientist says. Pleasure comes next, followed by desire, “so it is possible to like chocolate but not necessarily want it, for example when one has just eaten”. Pleasure and desire can be dissociated. Any food experience that triggers the release of dopamine associated with these three elements will act as positive reinforcement and influence future meals. It is a dysfunction of this reinforcement process that underpins most addictive behaviours, and notably drug use.
Triglycerides that mimic dopamine
How do nutrients activate this reward circuit? Serge Luquet’s team has found a partial response to the question by focusing on the action of the triglycerides that circulate in the blood after a meal. “We observed that triglycerides were able to communicate directly with the reward system. To understand why, we injected them directly into the carotid artery of mice to make sure they would reach the brain,” he explains. This modified the dietary behaviour of the rodents by reducing their natural tendency to prefer fatty and sweet foods. The animals subsequently displayed a preference for a more balanced diet, showing that the reward associated with such foods had diminished.
“In the brain, triglycerides are able to act like dopamine, in particular by reaching DRD2 neurons – which respond to this hormone – and reducing their activity,” notes Serge Luquet. By entering the brain, the lipids interact with the dopaminergic circuits, thus modulating the desire and pleasure associated with food. At least in mice.
In humans exposed to appetising smells (biscuit or strawberries), MRI imaging has shown activation of the reward system. But the brain’s response to these odours is much weaker just after eating. “Triglyceride levels in the blood are much higher after a meal, and we were able to demonstrate a strong correlation between elevated postprandial lipids and how the brain responds to a food trigger: triglycerides act as a signal in the brain to regulate the intake of ‘pleasurable’ foods.”
When this system is disturbed and the signal is poorly interpreted, dietary disorders ensue. Excessively high blood triglyceride levels could thus desensitise neurons to dopamine in the long term, thus altering their ability to generate a cerebral reward; this in turn will give rise to excessive food intake that compensates for this loss of pleasure.
This phenomenon is observed in some obese individuals: the hedonic signal of dopamine is lost, so overstimulation with food is a necessary compensation. A significant proportion of the population may have dysfunctional DRD2 neurons. Could abnormally high blood triglyceride levels be one of the reasons for this disturbance? That is what Serge Luquet’s team aims to investigate in the coming years.
Sugar addict?
Some scientists go even further and think that because of their action on the reward system, certain nutrients may have the same effect as addictive drugs. This is the case for Serge Ahmed, research professor and head of the Pathological Decision-Making in Addiction team at the Neurocampus4 in Bordeaux (southwestern France), who has been studying the addictive potential of sugar during the past fifteen years. “Already in 1838, Balzac was highlighting the harmful effects of sugar in his Traité des excitants modernes, comparing it with tobacco or alcohol,” Serge Ahmed points out. The first studies on the neurobiological effects of sugar came to light two hundred years later, in the early 2000s, “with work revealing that chronic sugar consumption in rats caused a state close to dependency, with clinical signs of withdrawal such as anxiety, diarrhoea or teeth grinding following its discontinuation.”

In 2007, the team observed that in the longer term, rats preferred sugar to cocaine. Since then, it has been demonstrated that sugar consumption activates the reward system by inducing dopamine release. Are we liable to become dependent on sugar, in the same way as heroine or tobacco? Not necessarily. “Among all cocaine users, 15% will be addicted. For sugar, the proportion ranges between 5% and 10%,” says Serge Ahmed. Individual vulnerability is linked to numerous environmental factors, such as past traumas, socioeconomic background or family eating habits.
Besides, is it sugar itself or a sweet taste that can make us addicted? “Studies have been performed in mice that were genetically modified to lose their taste receptors for sugar. Yet when they were fed sugar, activation of the dopaminergic network persisted. On the contrary, dopamine was not activated when artificial sweeteners were used,” explains the scientist. Nevertheless, if we accept that it is the ingestion of glucose that enhances our preferences, then sweeteners should cause no pleasure and “no-one would like sugar-free fizzy drinks!”. “In fact, sweeteners do not wipe out the hedonic effects of a sweet taste because as a general rule, they will be drunk during a meal. The brain will therefore have a sugar peak from the food ingested, plus the taste of the sweetener and be deceived,” analyses the scientist.
Excessive sugar consumption affects the brain in the long term
The fact remains that in the long term, chronic and excessive sugar consumption “can affect the functioning of the brain from a neurobiological point of view, having an impact on cognitive, motivational and emotional processes,” stresses Martine Cador, research professor and leader of the AddicTeam at the Aquitaine Institute for Cognitive and Integrative Neuroscience (INCIA).5 This is the case in particular when such consumption occurs during adolescence, at a time when neurons are maturing. “Mice that overconsume sugar in that period of life, at levels similar to those found in fizzy drinks, develop impaired motivation to find sugar in adulthood, a condition called anhedonia. It is as if the sweet sensation had become less pleasant,” suggests Martine Cador. Consumption at that age also increases the risks of depressive syndrome and reduces neurogenesis, or the formation of new neurons.
In Martine Cador’s view, the addictogenic profile of sugar nevertheless remains controversial: “cocaine multiplies the release of dopamine in the brain by 1000% or 2000%, compared with 50% or 100% for sugar; the activation kinetics are indeed very different. Sugar remains a natural reward.” Indeed, because the discovery of fruit trees was becoming increasingly rare for our ancestors, their brains were programmed to eat as much sugar as possible and store any excess in the form of fat. Under these conditions, no evolutionary pressure has had the time to teach the brain to limit our sugar intake! “This is the reason why the brain mechanisms that restrict it are extremely scarce; we are not neurobiologically equipped to control it,” concludes Serge Ahmed. Whether it comes to sugar or fat, our brain does not at present seem to be wholly adapted to our dietary environment.
Keywords
Share this article
Author
Léa Galanopoulo has a biology degree and is currently studying scientific journalism at Paris-Diderot university.
Comments
Log in, join the CNRS News community