You are here
The origins of the Standard Model
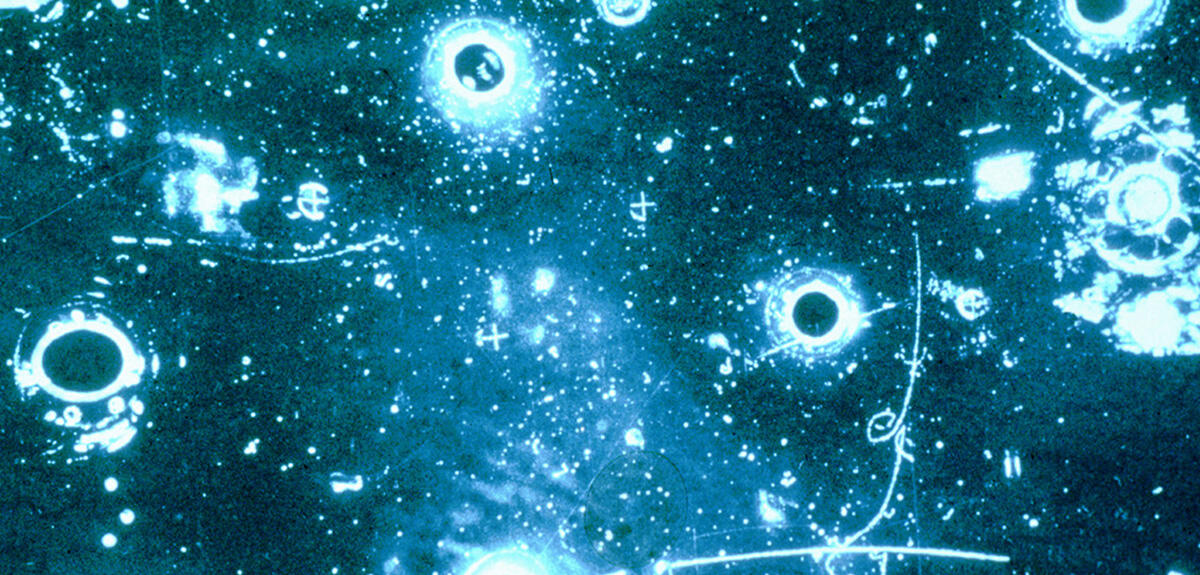
In 1973, André Lagarrigue’s team at CERN used the Gargamelle bubble chamber to demonstrate the existence of weak neutral currents. This result made it possible to unify, within a single theory, two fundamental interactions that had previously been thought to be distinct: the electromagnetic force, responsible for attraction and repulsion between electrically-charged particles; and the weak force, responsible for beta radioactivity. The development of this “electroweak” theory led, a few years later, to the establishment of the Standard Model, which was complemented in 2012 by the discovery of the Higgs boson, and describes all of the particles and forces that make up and govern known matter.
Beta decay and the weak force
The discovery of the weak “force” dates back to the 1930s, when the physicist Enrico Fermi proposed the existence of an interaction explaining beta radioactive decay, which appears in the atomic nucleus via the transformation of a neutron into a proton, accompanied by the emission of an electron and a neutrino. In the late 1950s, the developments of Fermi’s model postulated that this weak interaction was “carried” by a yet-unobserved mediating particle, called “W” for “weak.” According to quantum field theory, fundamental interactions between particles of matter (also called “fermions”) are “conveyed” by the exchange of other particles called “bosons.” The range of these interactions depends on the mass of the mediating particle: the greater its mass, the more limited the force’s range. The mediating particle for the electromagnetic force – the photon – has no mass, hence the infinite range of this interaction.

Fermi’s model nevertheless raised a major problem: “the mediating particle’s mass had to be high, in keeping with a force with a short range. Yet a non-zero mass would mean that the fundamental symmetry of the theory is broken, and the description of weak interaction via boson exchange could no longer work,” points out Claude Charlot, a research professor at the École Polytechnique’s Leprince-Ringuet Laboratory1.
Electroweak theory
The solution to this problem came in 1964, with the Brout-Englert-Higgs2 mechanism. “It is based on two elements: a new quantum field, known as a ‘Higgs field,’ and spontaneous symmetry breaking,” Charlot notes. Such symmetry breaking apparently occurred a few fractions of a second after the Big Bang, via the sudden change in the minimum energy state of the Universe: like a ball balancing on a pass between two valleys, and falling to one side or the other to reach a new balance at the bottom of one of the valleys. The introduction of the Higgs mechanism thus avoided challenging the mathematical symmetry of weak theory.
A few years later, Martinus Veltman and Gerard t’Hooft reformulated the model to obtain results with no infinites in the calculations. Relying on these calculations, Sheldon Glashow, Steven Weinberg, and Abdus Salam developed the electroweak theory. “Alongside electrically-charged W+ and W- bosons already predicted by earlier weak theories, electroweak theory added a third intermediary, the Z0 neutral boson,” explains Delphine Blanchard, a doctoral student in the history of science at the Centre Alexandre Koyré3. This hypothetical neutral boson’s existence should result in the observation of a “neutral current” in addition to the “charged currents” due to W+ and W- bosons.
The Gargamelle project
In the late 1960s, Lagarrigue proposed a series of experiments on weak interaction by exposing a beam of neutrinos and antineutrinos inside CERN’s Gargamelle’s bubble chamber. “The idea behind its construction dates back from the Sienna Conference in 1963. It measured 4.8 metres in length, 2 metres in diameter, and contained approximately 12 cubic metres of freon,” recalls Bernard Degrange, research professor emeritus at the CNRS. “The liquid has to be thinned to be in a pre-boiling state. The ionisation energy locally applied by a charged particle creates bubbles along its path. Flashes light up the inside of the chamber, allowing us to photograph the traces of charged particles with cameras,” Charlot points out.
Since neutrinos have no charge, they leave no trace in the chamber. However, they interact with the nuclei of the atoms that make up freon – carbon, fluorine, and bromine – by producing charged particles that can be detected. “Freon increases the target’s mass, and hence the probability of detecting neutrino interactions. It is also an effective way of identifying electrons through radiation, in addition to spotting – via their conversion into e+e- pairs – the gamma photons resulting from the interaction of neutrinos, or the decay of secondary particles. Finally, the average length of neutron interaction in freon is much smaller than the dimensions of Gargamelle, making it possible to detect their presence.”
Two types of sought-after events
The observation of neutral currents was only 8th on the list of things to do with Gargamelle. “During events in the bubble chamber, there were 8 stereoscopic views that allowed us to analyse the results from a number of different angles,” Degrange recalls. Beginning with the first experiment in the chamber in 1971, neutrino interactions producing a muon and an electron were observed, a signature of the charged current process. There remained 20-30% of events that did not include a muon or an electron in the final state, and could be the result of either two neutrons, or the recently predicted neutral currents. “That is why in 1971, we decided to also measure these events,” adds Degrange. Two types of event were especially sought: the interaction of a neutrino with an electron in a liquid, and the interaction of a neutrino with a hadron – a proton or a neutron.

Yet how could it be determined whether these were neutral currents or just the interaction of neutrons? “When the interaction of neutrinos produced a neutron outside the visible volume, the phenomenon was classified as a ‘neutral current candidate’. According to observations, there necessarily were neutral current neutrino interactions,” Charlot details. In July 1973, the researchers confirmed 166 hadronic events and one electron event. “That same month, Paul Musset announced the discovery at CERN, and papers for each type of event were sent to the scientific journal Physics Letters, published on 3 September 1973,” sums up Blanchard.
Emergence of the Standard Model
In 1974, the existence of neutral currents was confirmed by the Argonne group in the United States using a 12-foot hydrogen chamber, in addition to the Harvard-Pennsylvania-Wisconsin and CalTech experiments of Fermilab, which paved the way for the Standard Model of Particle Physics. The direct observation of W and Z bosons in 1983 by CERN’s UA1 and UA2 collaborations, which earned Carlo Rubbia and Simon van der Meer the 1984 Nobel Prize in Physics – and later that of the Higgs boson in 2012 at the LHC, which earned François Englert and Peter Higgs the same distinction in 2013 – complemented and definitively supported the model.

While it remains the best description of the subatomic world today, this Standard Model still has grey areas. For example, it does not explain mass scales for various generations of quarks and leptons, the origin of the Higgs field, or the nature of the dark matter postulated by astrophysicists to account for the formation and survival of galaxies and galactic clusters. So many mysteries that remain to be solved by the physics of the 21st century…♦