You are here
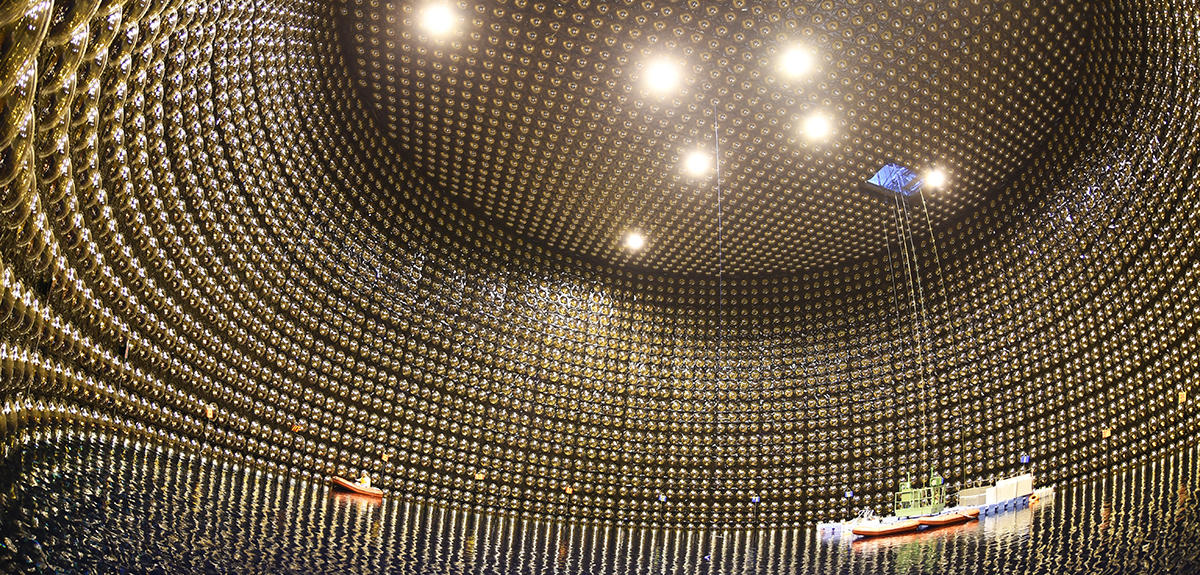
"Among the elementary particles that make up matter, neutrinos are the most mysterious. Better knowledge of their properties could pave the way for a new physics." Like many physicists, Christine Marquet from the Centre d’études nucléaires de Bordeaux Gradignan1 does not hide her fascination for neutrinos. Since their existence was predicted in 1930, and then confirmed in 1956, scientists have conducted a great many experiments and revealed the numerous properties of these particles. Yet a series of questions still surrounds them today, whose answers promise to revolutionize our knowledge of matter and the Universe.
Neutrinos can be produced in the cores of stars during nuclear fusion reactions, or in the atmosphere from the collision of cosmic rays (high energy particles from space). They are both highly abundant but also very “discreet,” because they interact so little with matter that they pass through everything in their path. For instance, billions of them pass through us every second without producing the least effect, and only one neutrino out of ten billion crossing through the Earth succeeds in interacting with an atom.
This distinctive feature, combined with an extremely low mass and lack of electric charge, has long made neutrinos some of the most elusive particles around. To capture them, physicists have had to build giant sensors made of thousands of tons of a target material, most often a liquid. The Super-Kamiokande detector in Japan, currently the world's largest, measures 40 meters high and 40 meters in diameter, and contains 50,000 tons of water. Detection takes place indirectly when a neutrino interacts with an atom in the water and produces charged particles, which in turn emit light. It is this light that thousands of sensors record in the reservoir.
The Particle with Three-flavors
We know that neutrinos exist in three different states, or "flavors" as physicists call them: electron, muon, and tau. Surprisingly, they are able to spontaneously transition—or mutate—from one flavor to another as they move, and are one of the few elementary particles endowed with this property, known as "oscillation."
This phenomenon was demonstrated by the Super-Kamiokande detector in 1998, when it observed neutrinos from the atmosphere, and by the Sudbury Neutrino Observatory (SNO) in Canada in 2001, when it observed neutrinos from the Sun. Records showed that a certain type of neutrino was lacking from these two sources in comparison to what was predicted by theory. The results showed that neutrinos did not actually disappear, but rather mutated from one type to another before arriving on Earth. Many other experiments followed, including some in France, all of which confirmed the phenomenon. This time, detectors captured neutrinos that were not natural in origin, but rather produced by nuclear reactors as part of their normal functioning, along with neutrinos emitted by particle accelerators. By verifying the source in this way, it became possible to more precisely measure the parameters of these oscillations, especially the probability that a neutrino will change from one flavor to another at a given moment.


The key finding of these experiments was that this oscillation of neutrinos can only be explained if they have a mass. This is a major surprise, because "according to the Standard Model, the conceptual framework that describes particles and their interactions, neutrinos are not supposed to have one. It's a weakness in the theory, a sign that we must revisit our theory, or at least supplement it," observes Michel Gonin, a researcher at the Leprince-Ringuet laboratory.2
Many theoretical extensions have already been proposed to explain the origin of the neutrino’s mass, and to understand why they are so small, since their mass values are at the most one millionth that of an electron. Theorists are outdoing one another—in terms of imagination—to find alternatives to what is known as the "Higgs boson" mechanism discovered at the LHC in 2012, which provides mass for other particles, but does not work for neutrinos. To decide among these different scenarios, it is necessary to establish the exact mass of the neutrino, which remains unknown today. "The experiments that focus on oscillations have measured the difference between the masses of three types of neutrinos, but not the absolute mass of either of them," points out Dominique Duchesneau from the Laboratoire d’Annecy de physique des particules (Lapp).3
To determine this mass, physicists must take an interest in another process, the "beta" radioactive decay of certain atomic nuclei, tritium in particular. During the reaction, a neutrino and an electron are emitted, with the latter providing almost all the energy coming from the transformation of the nucleus. The key challenge is to precisely measure the electron’s energy, and to compare it to the energy of the decay, with the tiny difference corresponding to the mass of the neutrino. For now, no experiment has achieved the level of precision needed to measure this mass, although physicists are working relentlessly to improve the sensitivity of their instruments.
What About Antineutrinos?
Another question raised by neutrinos that also threatens the Standard Model is whether neutrinos have their own antiparticle. Physicists are well aware that each particle of matter can be associated with a particle of antimatter—a mirror image—with the same mass but with an opposite electric charge. Hence, during certain phenomena, antielectrons with a positive charge are created at the same time as electrons with a negative charge. But as neutrinos have no electric charge, this raises the question of whether a neutrino and an antineutrino are one and the same. If this were the case, it would be the only particle of matter to possess such a property, which would subsequently need to be explained.
To verify this hypothesis, physicists once again relied on a process of atomic decay, but one that is much more rare than beta radioactivity, known as "double beta" decay. This time, two electrons and two neutrinos are emitted during the reaction. Researchers are actually trying to observe a particular type of this process: a double beta decay in which no neutrinos are emitted. Paradoxically, the existence of this phenomenon would be indisputable proof that the neutrino is its own antiparticle. A number of teams are pursuing this challenge, unsuccessfully for the moment, although that has not discouraged physicists, who are already preparing what comes next.
In France for instance, a new detector named SuperNemo is being built at the Laboratoire souterrain de Modane, with the involvement of CNRS researchers. It will take over from NEMO3,which was in operation from 2003 to 2011. "To give us a better chance of observing this decay, we increased the size of the radioactive source from 10 to 100 kilograms in its final version. We also considerably reduced the residual radioactivity emitted by the detector's material to prevent it from distorting the measurements, and we improved the precision for measuring the energy of the particles that are to be detected," explains Christine Marquet, the spokesperson for the SuperNemo international collaboration.
Sterile Neutrinos
The list of neutrinos' peculiarities does not end there. A few years ago, a deficiency was observed in the flow of neutrinos produced by nuclear reactors. A number of theories were proposed to account for it, including one that was particularly intriguing: the presence of a fourth type of neutrino in addition to the three other flavors.
With a much greater mass than the others, this new neutrino does not interact with ordinary matter, hence its name of "sterile" neutrino. The deficit observed would subsequently be explained by the fact that certain neutrinos are transformed into sterile neutrinos, which remain undetectable. Sterile neutrinos offer other qualities to theorists as well. They could intervene in certain mechanisms to explain why neutrinos are so light. In addition, because it has a large mass, it could also be a candidate for dark matter, the component that represents over 25% of the Universe, but whose nature remains unknown.
Here again, the presence of a fourth neutrino would be a unique case in the world of elementary particles, further confirming the key role of neutrinos in exploring new territory in physics. Yet it is important to remain prudent. "A simpler explanation would be that the model representing the fission process in a reactor, which is used to calculate the flow of neutrinos, presents an error," adds Duchesneau. A number of experiments, including Stereo in France and SoLid in Belgium, both of which involve CNRS researchers, are looking closely at this phenomenon. Within the next two years, they should be able to indicate whether to continue pursuing the possibility of sterile neutrinos.
In addition to challenging the current foundations of particle physics, neutrinos could also explain one of the greatest mysteries, namely why the Universe is made solely of matter and not antimatter. During the Big Bang equal quantities of matter and antimatter were supposed to have been created, before their mutual annihilation and disappearance in a surge of energy. Something tipped the scales in one direction. According to physicists, this primordial imbalance should manifest itself in the form of different behavior between particles of matter and particles of antimatter, which is called the "violation of CP symmetry." "This violation has already been observed for quarks, but it is not enough to explain the initial imbalance. This would be different with neutrinos, as measurement of oscillations suggests that they could generate enough violation to solve the mystery," enthuses Anatael Cabrera from the laboratoire Astroparticule et cosmologie.4 Nevertheless, this phenomenon still has to be demonstrated.
That is precisely the goal of two experiments currently underway: T2K in Japan and Nova in the US, which seek to observe differences in how neutrinos and antineutrinos oscillate. To do so, they use a particle accelerator to emit a beam of neutrinos of a particular flavor, muon neutrinos, at a detector (Super-Kamiokande for T2K) located a few hundred kilometers away (300 kilometers for T2K, 800 for Nova). Researchers count the number of neutrinos that transform into electron neutrinos, and then repeat the experiment with muon antineutrinos.
T2K has provided the most promising results to date. "The difference between the number of neutrinos and antineutrinos that we detected leans toward a strong violation of CP symmetry. But the results are not significant enough yet, it could simply be a statistical fluctuation," observes T2K member Michel Gonin.
Two New Pioneering Experiments
Physicists would need to record many more events before any talk of a discovery, but current instruments are not sufficient. Scientists have grasped this, and are already preparing the next generation of experiments, which will be equipped with a more intense beam to produce more neutrinos, as well as a larger detector to detect more of them. That would be Dune, which is under construction in the US, and Hyper-Kamiokande in Japan, which should be greenlit for approval at the end of the year. They each bring together a thousand researchers from different countries, and represent pioneering experiments in the field of particle physics.

Hyper-Kamiokande will use the same detection technique as its predecessor, but with a volume of water ten times greater. Dune will implement a technology that has been used previously, but never on this scale, with a detector containing 40,000 tons of liquid argon. When a neutrino collides with an argon nucleus, charged particles will be produced, which will in turn ionize the argon. The electrons created during this ionization will then be detected, enabling the three-dimensional reconstruction of the trajectory travelled by the charged particles. "The technique will function at -185 °C, the temperature of liquid argon, and will provide a very precise photograph of the movement of all particles, thereby revealing the presence of neutrinos," enthuses Dario Autiero, a member of Dune and l’Institut de physique nucléaire de Lyon.5
In addition to Dune and Hyper-Kamiokande, a third experiment will prove crucial to reach the precision needed to demonstrate the violation of CP symmetry: the Juno experiment, which is currently under construction in China, and also involves the participation of CNRS researchers. With its giant spherical reservoir measuring 35.4 meters in diameter and filled with 20,000 tons of a benzene-based liquid, Juno will analyze the oscillation of neutrinos emitted by two nuclear reactors, both located exactly 53 kilometers away. This will be the world’s first experiment using the same detector to characterize both solar oscillations (the same type of neutrinos as those generated by the Sun) and atmospheric oscillations (the same as those observed for neutrinos coming from the atmosphere). If all goes well, beginning in 2021, Juno will identify the parameters of these oscillations with unprecedented accuracy. “Such precise measurements will serve as an invaluable reference for later experiments trying to discover CP violation,” comments Cabrera, the scientific manager of Juno for France.
Carrying on the work of Juno, Dune and Hyper-Kamiokande, which are slated to start in 2026, should reveal the presence of the famous violation of symmetry after a decade or so of measurements. Thanks to neutrinos, physicists have never been closer to solving the mystery of antimatter.
_______________________________________________________________
Neutrinos, precisous cosmic messengers

Studying the properties of matter is not the only thing for which physicists use neutrinos. They are also prodigious messengers for elucidating the most violent events in the Universe, such as the ejection of matter by supermassive black holes, collisions between neutron stars, or the explosion of supernovas. As they are neither absorbed nor deviated by the intergalactic environment, the cosmic neutrinos produced during these cataclysms make it all the way to the Earth, thereby directly attesting to these phenomena and proving of great interest to astronomers. They are more rare and possess more energy that the neutrinos produced by the Sun or the Earth's atmosphere, in addition to being more difficult to capture. To do so, scientists have launched the construction of large detectors that will serve as a sort of neutrino telescope. In France, the Antares instrument located on the sea floor 2,500 meters below the Mediterranean, off the coast of Toulon, includes nine hundred sensors divided across twelve lines. Since 2008, it has been scanning the passage of neutrinos in the water across a surface of 10 hectares. Beginning in 2022, its successor KM3NeT, which is under construction at three different underwater sites (one near Antares, another in Sicily, and a third one in Greece), will observe a volume of 5 cubic kilometers. Even so, the large telescopes devoted to observing neutrinos will focus on more than their astronomy, as the future neutrino detectors Dune and Hyper-Kamiokande will be used for more than just particle physics. By virtue of their great size, they will also be able to detect cosmic neutrinos, and help shed light on the most violent events in the Universe.