You are here
Fusion in stars reproduced in the lab
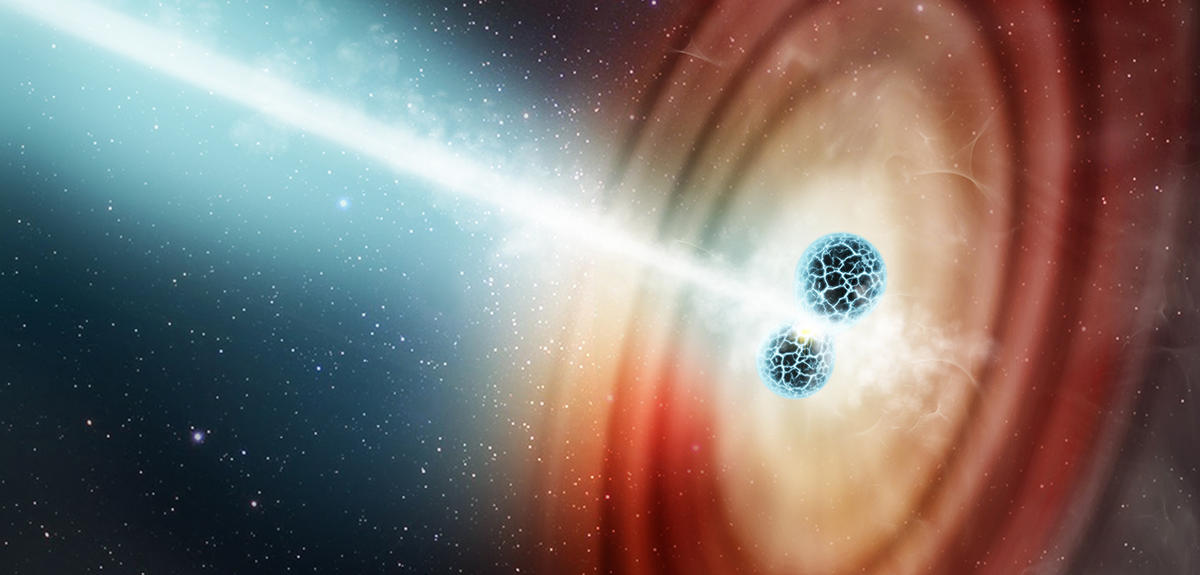
You're a nuclear physicist and you have a special interest in fusion in stars. Why is that?
Sandrine Courtin:1 The birth of a star results from matter collapsing in on itself under the effect of its own gravity. Once a certain density and temperature are reached, fusion reactions between the atomic nuclei present in the core start to take place, releasing a huge amount of energy, counteracting gravity and creating a state of equilibrium that lasts as long as the star still has ‘fuel’. Once this runs out, its life comes to an end either by collapsing in on itself or following different scenarios that depend on its characteristics, such as its initial mass. Understanding the fusion reactions that occur in stars is therefore essential to comprehending how they evolve.

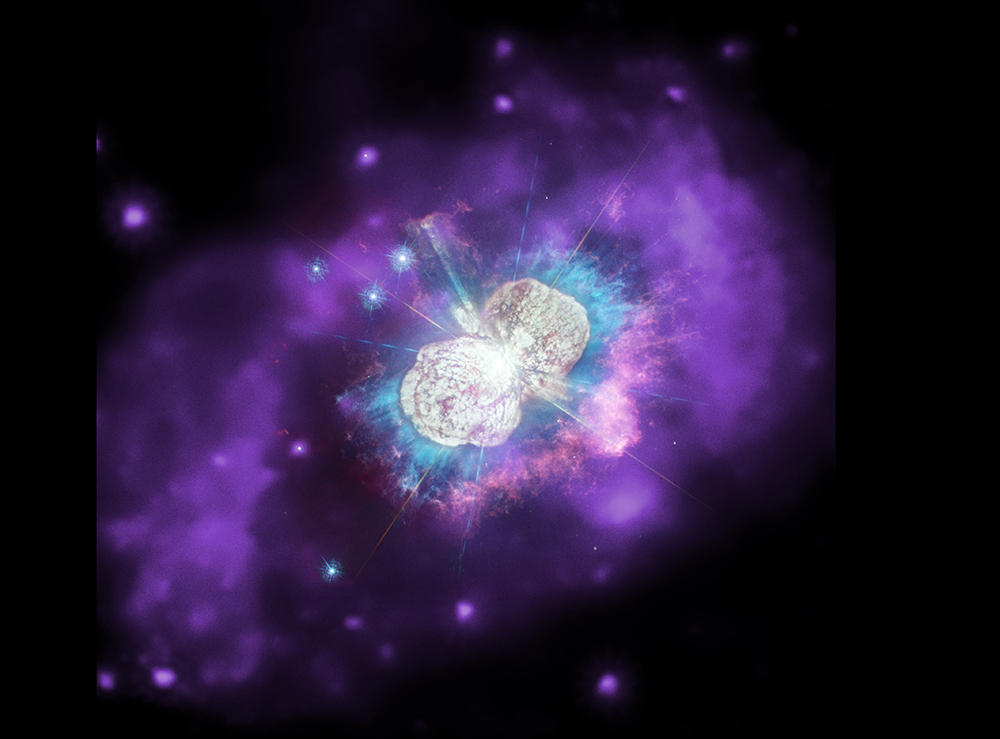
This understanding is also the key to explaining the abundance of the different elements in the Universe, isn't it?
S. C.: That's right. At the time of the Big Bang, primordial nucleosynthesis gave rise to hydrogen, a little helium and trace amounts of lithium. The next elements in the periodic table, at least up to iron and nickel, were produced in fusion reactions inside stars. Still heavier elements result from the even more energetic processes in neutron stars or supernovae, for example. In any case, to understand the fusion reactions in stars is to understand the origin of the elements, especially carbon and oxygen, which are essential for life.
What exactly is a nuclear fusion reaction?
S. C.: It’s a reaction between two atomic nuclei which, after colliding, fuse, forming other elements. For such reactions to take place, the original nuclei must have enough kinetic energy to overcome the electrostatic repulsion between them. Once this barrier has been crossed, the strong nuclear interaction, which is a short-range attractive force, takes over, bringing about fusion.
It is worth noting that the temperature inside stars isn’t high enough to provide the nuclei with the kinetic energy that, according to classical physics, they would need in order to overcome the electrostatic barrier that prevents them from fusing. However, fusion does nonetheless happen due to a purely quantum effect, the tunnel effect, which allows particles to break through the barrier. The probability of the tunnel effect occurring is extremely low. Inside stars, however, this is counterbalanced by the sheer numbers of particles involved. It is these so-called ‘low-energy’ nuclear reactions that I focus on.

Which ones exactly?
S. C.: In the last few years, I've carried out a series of experiments aimed at determining the probability, depending on the energy involved, of the fusion of two carbon atoms. This reaction, which takes place in stars with masses typically greater than eight solar masses, is extremely important for at least two reasons. Firstly, it enables us to determine the abundance of carbon in the Universe. In stars, this element is both created by the fusion of three helium nuclei via a resonance – and destroyed, either by the fusion of two carbon nuclei or that of a carbon and an oxygen nucleus. Therefore, the abundance of carbon ultimately depends on the balance between these different reactions. And secondly, the carbon-carbon reaction is, in a way, the gateway leading to the creation of all the other elements of the periodic table.
Does this mean that the properties of the carbon-carbon reaction were little known until now?
S. C.: They can in principle be determined using quantum theory. This sometimes results in equations that are extremely difficult to solve, forcing theorists to proceed step by step on the basis of hypotheses such as the existence of clusters in nuclei, yielding results that still need to be substantiated. The other possibility is to reproduce the conditions inside stars in the laboratory, and directly measure the probability of a particular reaction occurring.
That doesn't sound easy!
S. C.: You're right! These experiments are carried out in an accelerator. They involve firing a beam of carbon nuclei at a target made of the same element and measuring the characteristics of the fusion products, from which we can determine the properties of the reaction itself. When two carbon nuclei fuse they form a nucleus of magnesium that rapidly decays either to a nucleus of neon, one of helium (also called an alpha particle) and high-energy photons (gamma rays), or alternatively to a sodium nucleus, a proton, and gamma rays.

Over the past fifty years or so, experimenters have attempted to determine the properties of the initial fusion by measuring either the particles (alpha particles and protons), or the gamma rays. The problem is that the probability of a carbon-carbon reaction occurring is so low that the signals associated with it were drowned out in intense experimental noise, resulting in significant measurement uncertainties. Specifically, at the energies relevant to astrophysics, the dominant signal turns out to be produced by fusion of carbon nuclei with deuterium nuclei from the extremely low residual humidity present in the experimental facilities, even though they are under a vacuum!
So it was in this context that you came up with an innovative experiment?
S. C.: The challenge is to choose the appropriate experimental events to record. On paper, the best way of doing this is to simultaneously measure the particles and the gamma rays when they are ‘in coincidence’. This ensures that the whole event is actually produced by the reaction being studied, and not by a side reaction. The problem was that no one had ever done this, since there was a risk of eliminating so much of the signal that there would be nothing left to measure. Our bold move was to realise that, if we set up optimal experimental conditions and used intense beams, it would be possible to collect data. In 2014, we tested the idea with an American research team using the accelerator at Argonne National Laboratory, near Chicago (US). The results we obtained convinced us that it would be well worth setting up a dedicated trial.
And that turned out to be no easy matter.
S. C.: Indeed! We worked out that we would have to collect accelerator data for several months, whereas a standard nuclear physics experiment typically lasts just one week. As luck would have it, a new particle accelerator was about to become operational at the Laboratory of the Physics of the two Infinities Irene Joliot-Curie (IJCLab),2 near Paris, which expressed interest. At the same time, I asked colleagues at the UK universities of York and Surrey whether they would be interested in a collaboration involving their gamma-ray detectors, to which they agreed. There was some urgency, because in an ultra-competitive field like this, our preliminary results had whetted appetites!

What followed was STELLA, a remarkable international collaboration involving outstanding researchers, engineers and technicians from the laboratories of the CNRS's National Institute of Nuclear and Particle Physics (IN2P3), the Strasbourg-based Hubert Curien Pluridisciplinary Institute (IPHC), in northeastern France, and universities in the UK and the US.
What were the main challenges for STELLA?
S. C.: Overall, this was an experiment where everything was pushed to the limit. In this type of trial, beam intensity is generally in the region of a nanoampere. But in our case, it was 1,000 times greater. For our 100-nanometre-thick targets to resist, we had to cool them down by mounting them on a wheel rotating at 1,000 revolutions per minute. To reduce noise, we also paid special attention to the quality of our vacuum as well as to our trigger electronics. Finally, to analyse the data we relied on methods used in particle physics, a field in which signals often have to be extracted from extremely intense experimental noise.
What were STELLA's findings and what will they change?
S. C.: Over the whole energy range corresponding to the conditions found in stars of between 12 and 25 solar masses, we have achieved unprecedented precision. This will have repercussions on the results of stellar evolution models, which until now have been based on flawed theoretical data. Specifically, the new parameters we have measured for the carbon-carbon reaction have implications for the formation of aluminium, barium, and strontium in stars. They may also alter the end-of-life scenarios of some stars. These analyses are under way, in particular with our colleagues from the University of Geneva (Switzerland).
What are the next steps?
S. C.: After studying the carbon-carbon fusion reaction, our rapidly-growing team at the IPHC (a new researcher is joining us this year) intends to tackle the carbon-oxygen reaction, which involves firing a beam of oxygen nuclei at a carbon target. This reaction is harder to analyse since there is a wider variety of decay pathways than in the carbon-carbon reaction. Then, in five to ten years’ time, we hope to study the oxygen-oxygen fusion reaction, which is much trickier to carry out experimentally and for which there is currently no really consistent data. It’s early days yet!
Author
Born in 1974, Mathieu Grousson is a scientific journalist based in France. He graduated the journalism school ESJ Lille and holds a PhD in physics.